The Chemical Structure of DNA: An In-Depth Analysis
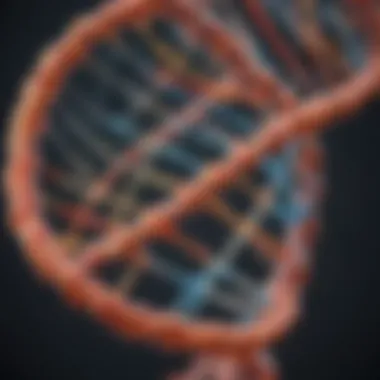
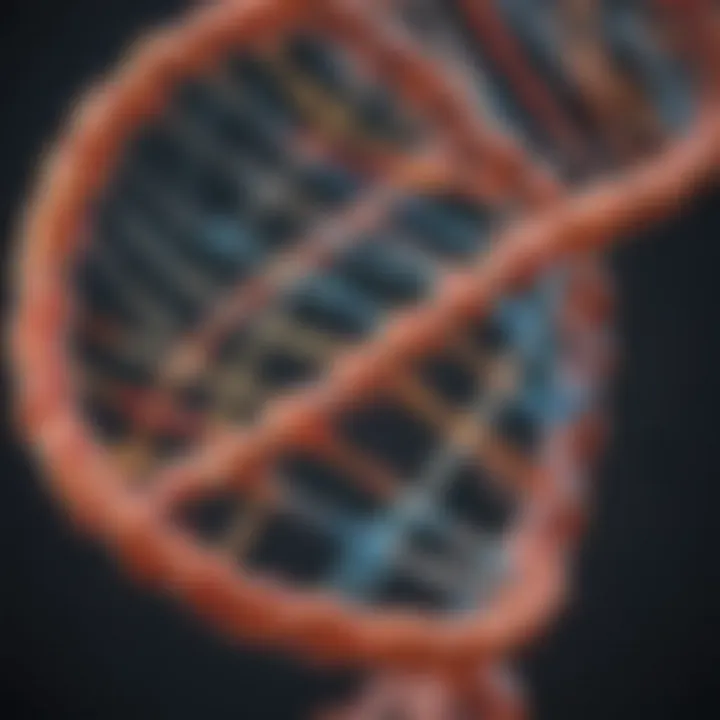
Intro
Understanding what makes life tick has always held a certain allure. At the heart of this fascination lies DNA, the specter of life’s instructions packed tightly into what seems to be an ordinary molecule. But don’t let its unassuming appearance fool you; this structure is a treasure trove of information about how organisms grow, develop, and function.
DNA, or deoxyribonucleic acid, is not merely a strand of molecules; it is the blueprint of life. Every living organism, be it a tiny bacterium or a towering redwood, utilizes DNA to carry the genetic code that determines its existence. As we peel back the layers of DNA’s chemical structure, it becomes clear that its elegance is not just in its design but also in its profound implications for genetics and biology as a whole.
When we dive into the depths of DNA, we encounter its double helix structure which truly sets it apart. This iconic shape, resembling a twisted ladder, is critical for its function, safeguarding the genetic information while allowing for replication and repair. The journey of this article will take us from the building blocks of DNA to the intricate dance of relationships between molecules, providing insight into how these components come together to create the very essence of life.
It's essential to explore the reasons behind studying DNA's chemical structure — not just for academic pursuit, but for its practical implications in fields like medicine, forensic science, and biotechnology, to name a few. With advancements in technology continually reshaping our understanding, we must stay abreast of the latest studies and techniques that unveil new dimensions of this ancient molecule.
The intricate discussions here will unravel key aspects of DNA structure, pivotal in both the realms of science and society. With a thorough comprehension of DNA's chemical underpinnings, one gains valuable insights into genetic modification, hereditary diseases, and evolutionary biology, demonstrating just how foundational this topic is to the life sciences.
Let's embark on this exploration—and uncover the depth of what DNA actually encompasses.
Intro to DNA
The world of molecular biology hinges on an intimate understanding of deoxyribonucleic acid, commonly known as DNA. This inherent importance is due, in part, to DNA's role as the foundational molecule that encodes genetic theory comprehension. Ranging from classical genetics to cutting-edge genomic technologies, understanding DNA is like holding the key to the treasure chest of life itself. Knowing its structure, composition, and function is critical for various scientific fields, including genetics, biochemistry, and even medicine.
One may ask, why does it matter so much? The significance of DNA lies in how it governs hereditary information. Without grasping the basic components and how they intertwine, the door to understanding genetic diseases, evolutionary biology, and biotechnology remains firmly shut.
In this exploration, blurring the boundaries between physics and biology emerges as a theme, demonstrating how DNA's structural resilience contributes to the stability of genomes and the specific encoding of information.
Definition of DNA
DNA is a molecule composed of two long strands of nucleotides that coil around each other, forming the well-known double helix shape. These nucleotides consist of three components: a phosphate group, a sugar molecule, and a nitrogenous base. The four bases—adenine, cytosine, guanine, and thymine—pair in specific combinations, with adenine always pairing with thymine, and cytosine with guanine.
This structural arrangement is not just an aesthetic affair. It provides the basis for genetic coding and the transmission of hereditary traits. Think of it as a sequence of letters in a book where not only the letters but also the order in which they appear creates meaning.
Historical Context
The journey to uncover the secrets of DNA began long before scientists understood its full implications. The 19th century saw the identification of nucleic acids in cellular material, although their significance remained largely unrecognized until the early 20th century. Rosalind Franklin’s X-ray diffraction images in the early 1950s contributed to the conclusive evidence for the double helix model, but it was James Watson and Francis Crick who, in 1953, presented this structure as a pivotal breakthrough.
Years of accumulated knowledge from the fields of zoology, chemistry, and physics converged to illuminate this molecular marvel. Each step of the investigative journey laid the groundwork for profound advancements in understanding cellular processes, ultimately leading to the mapping of the human genome. We now realize how DNA not only instructs life's processes but is also tightly entwined with the fabric of what it means to be human.
"Deoxyribonucleic acid is really a message embedded deeply within the structure of our cells; it carries with it the signatures of our history and futures."
As such, comprehending DNA is more than a simple academic exercise; it's about connecting with the mechanisms of life and the very essence of existence.
Molecular Composition of DNA
Understanding the molecular composition of DNA is crucial in grasping the intricate workings of life at a cellular level. Here, we dive into the fundamental elements that construct this marvelous macromolecule. The richness of DNA’s composition, from its basic nucleotides to the backbone structure, directly influences its stability, replication, and overall functionality. Researchers and students alike can benefit immensely from exploring how these components interact, leading to the grand architecture of genetic material. With a closer look at the structure, it becomes clear that every bit counts.
Nucleotides: The Building Blocks
At the heart of DNA’s structure lay the nucleotides. These are the building blocks that pair up in various combinations to encode the genetic information essential for life. Each nucleotide consists of a sugar, a phosphate group, and a nitrogenous base. The interplay of these elements forms the very foundation of DNA.
Adenine
Adenine is one of the four primary bases that make up nucleotides. It pairs with thymine in the DNA structure, forming a bond that is both stable and essential for proper genetic encoding. A key characteristic of adenine is that it possesses a structure that is rich in nitrogen, allowing it to form hydrogen bonds with thymine effectively. This makes adenine not only a fundamental part of DNA’s architecture but also a favorable choice in the realm of molecular biology. Its unique feature is the presence of an amine group, which can link with other components to facilitate various biological processes. However, there are some challenges in reactivity under specific conditions.
Cytosine
Cytosine stands out as a crucial player in the nucleotide assembly. It pairs specifically with guanine, contributing to the stability of the DNA molecule through three hydrogen bonds. Cytosine’s structure includes an amine and a carbonyl group, forming the foundation for its unique reactivity and complementarity. Its ability to engage in such bonding makes it an essential component for genetic fidelity. In some instances, the metabolic processes can subject cytosine to deamination, leading to potential mutations if not properly repaired.
Guanine
With its stimulating pairing with cytosine, guanine plays a significant role in defining the structure of DNA. Its molecular structure is distinguished by a fused ring system that not only enhances the capacity for hydrogen bonding but also contributes to its ability to maintain structural integrity under varying conditions. Guanine is often described as beneficial to the overall stability of the DNA helix due to its triple hydrogen bond with cytosine. However, its propensity to form other structures under certain environmental conditions can also lead to unwarranted changes in genetic information, which raises questions about its reliability.
Thymine
Completing the quartet, thymine is the base that pairs with adenine, forming the complementary base pair that is vital for DNA’s double helix. Thymine's molecular structure is similar to cytosine, yet it lacks the amine group, making it distinctly different. This apparent simplicity does not diminish its importance; thymine's presence ensures proper pairing and stability of the double helix. One unique feature is its role in DNA repair mechanisms—particularly in excising mismatched bases. However, modifications such as methylation can occasionally cause complications in gene expression and regulation.
Phosphate Backbone
The phosphate backbone is a critical part of DNA, providing structural support and facilitating the overall stability of the double helix. Composed of alternating phosphate groups and deoxyribose sugars, it creates a strong, stable framework for the genetic material. This backbone is highly polar and negatively charged, which influences how DNA interacts with proteins and other cellular machinery. The backbone’s resilience is paramount in protecting the delicate bases that encode genetic information from environmental damage.
Sugar Molecule: Deoxyribose
Deoxyribose, a five-carbon sugar, forms the other half of the backbone structure alongside phosphates. Its specific configuration—devoid of an oxygen atom on the second carbon compared to ribose—gives DNA certain stability benefits. This omission means that DNA is less reactive than RNA, which is pivotal for its role as the long-term storage medium for genetic information. Additionally, the structure of deoxyribose allows for directional polarity in the DNA strands, aptly enabling the formation of base pairs and ensuring accurate replication processes.
Arrangement of DNA Structure
The arrangement of DNA structure is a fundamental aspect of molecular biology, crucial for understanding how genetic information is stored, replicated, and transmitted across generations. The meticulous organization of DNA not only safeguards its integrity but also plays a pivotal role in various biological mechanisms. This section provides insights into the architecture of DNA, focusing on its double helical nature and the orientation of its strands, which together facilitate essential processes such as replication and gene expression.
The Double Helix Concept
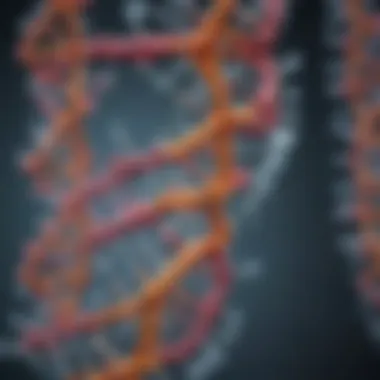
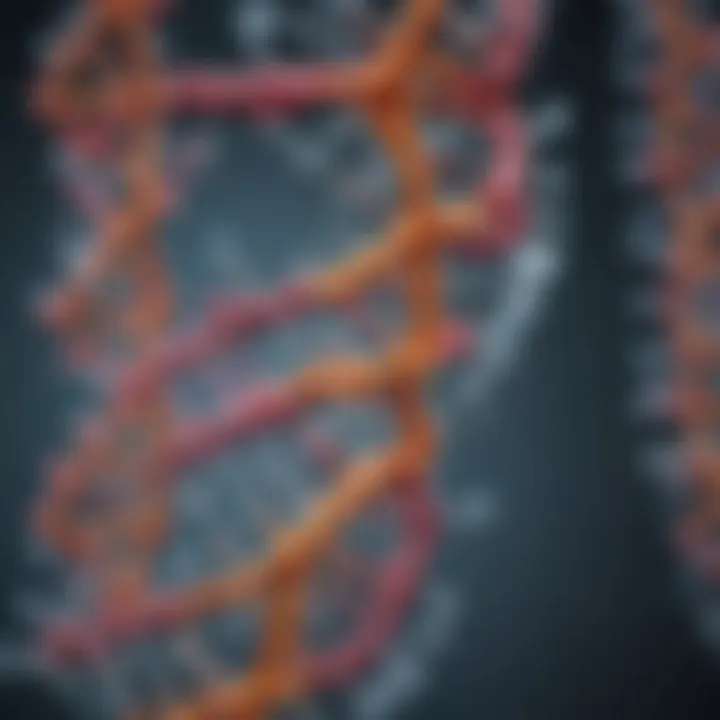
At its core, the double helix represents a stunningly elegant solution to the challenge of packing long stretches of genetic material into a compact form, while still allowing access to this information when needed. The double helix, first described by Watson and Crick in 1953, consists of two strands twisted around each other, resembling a spiral staircase. This arrangement is not merely aesthetic but serves multiple critical functions in biological systems.
Structural Features
- Base Pairing: The rungs of the helical staircase are made of nucleotide bases—adenine, thymine, cytosine, and guanine—pairing specifically (A with T, C with G) through hydrogen bonds. This complementary base pairing not only supports the integrity of the structure but also ensures that genetic information is preserved during replication.
- Directionality: Each strand has a specific direction due to its sugar-phosphate backbone, created by the arrangement of its components. This characteristic allows for the efficient replication where DNA polymerases synthesize new strands by following an existing template.
- Minor and Major Grooves: The twisting of the strands leads to the formation of minor and major grooves, essential sites for protein interaction. Many proteins recognize specific DNA sequences by binding to these grooves, thus influencing gene regulation and expression.
- Supercoil Formation: The double helix can further coil upon itself to form supercoils, enhancing compactness in cellular environments—this structural adaptability is vital for eukaryotic organisms, where DNA is packed tightly into chromatin.
The double helix is more than a structural marvel; it's a dynamic entity that enables life's complexity by allowing for both stability and flexibility in genetic processes.
Antiparallel Strands
An essential feature of the DNA double helix is that the two strands run antiparallel to each other. This means that they are oriented in opposite directions, which is pivotal for various biochemical functions.
- Synthesis of New Strands: During DNA replication, enzymes like DNA polymerase can only synthesize new strands in a 5' to 3' direction. This creates a necessity for antiparallel alignment, as one strand serves as a leading strand and the other as a lagging strand. The lagging strand is synthesized discontinuously in short segments called Okazaki fragments.
- Strand Stability: The antiparallel arrangement contributes to the stability of the overall structure. The strand orientations allow the backbones to pair their phosphodiester linkages, reinforcing the integrity of the helix.
- Facilitating Repair Mechanisms: The antiparallel nature also plays a role in DNA repair syntheses. When damage occurs, the inverse orientation allows specialized enzymes to quickly locate complementary sequences and effectively repair the damaged portion.
In summary, the arrangement of DNA strands, particularly the double helix concept and their antiparallel nature, unravels a sophisticated design that is fundamental to its function. Understanding these aspects lays the groundwork for further exploration of DNA's role in genetics, from replication to repair mechanisms, ultimately framing a larger narrative of biological inheritance.
Base Pairing and Hydrogen Bonding
Base pairing and hydrogen bonding serve as the cornerstone for the integrity and functionality of DNA. At the heart of the structure of deoxyribonucleic acid lies a delicate yet robust network of these interactions. Understanding how these mechanisms work informs not only the genetic blueprint of organisms but also the processes that enable cellular functioning day-to-day.
Complementary Base Pairing
Complementary base pairing refers specifically to the pairing of nucleobases on opposite strands of the DNA double helix. Each base—adenine (A), thymine (T), cytosine (C), and guanine (G)—is attracted to a partner on the opposing strand: A pairs with T, and C pairs with G. This selective pairing is not merely incidental; it is fundamental to the fidelity of DNA replication and transcription. The specificity of these interactions ensures that genetic information is passed on accurately from one cell generation to another, enabling the complex processes of life.
Here are a few points to consider about complementary base pairing:
- Genetic Diversity: Mutations can occur when there are mistakes in base pairing, leading to variations in the genetic code.
- Evolutionary Implications: Base pair similarities and differences can be indicative of evolutionary relationships among species.
- Biotechnology Applications: Understanding these pairings has enabled advances in gene editing technologies like CRISPR.
In practice, the stability of base pairing is often illustrated through Chargaff's rules, which state that the amount of adenine equals that of thymine, and the amount of cytosine equals that of guanine. This kind of symmetry is more than academic; it’s a foundational concept that underpins biological diversity and inheritance.
Hydrogen Bonds: Stability and Structure
The physical links between these complementary bases are not covalent bonds but rather hydrogen bonds. This choice of bonding may seem fragile, yet it lends remarkable stability to the overall structure of DNA. Each A-T pair forms two hydrogen bonds, while the more complex C-G pair forms three. This difference is significant, as it influences the melting point of DNA, affecting how tightly or loosely the strands hold together under various conditions.
To identify some key roles of hydrogen bonds:
- Structural Integrity: They help maintain the double helical structure, providing the necessary tension for the DNA molecule.
- Reversible Interactions: Hydrogen bonds allow for the separation of DNA strands during replication and transcription, a function crucial for cellular processes.
- Facilitation of Enzyme Action: Specific enzymes identify and interact with these bonds, facilitating repair and other critical functions in the cell.
"Base pairing and hydrogen bonding are not just supporting players in the narrative of DNA—they are central characters, dictating how the story of life is encoded, replicated, and expressed."
The combination of base pairing and hydrogen bonds creates a unique set of rules governing the behavior of DNA. Understanding these interactions is vital for any deeper exploration of genetic mechanisms, and it sets the stage for investigating how alterations can lead to diseases or other phenomena.
Functional Implications of DNA Structure
The unique structure of DNA is not just a marvel of biology; it has profound implications for how genetic information is stored and processed. Each twist and turn of the double helix plays a crucial role in its functionality. Understanding the implications of this structure offers meaningful insights into genetics, cellular processes, and even the evolution of life itself. Here, we shall explore two pivotal aspects: how DNA functions as a repository of genetic information and how it directly influences the synthesis of proteins.
Role in Genetic Information Storage
The primary function of DNA is to store genetic information. Think of DNA as a highly sophisticated library, where each chapter is a gene that contains instructions for building proteins. This information, encoded in sequences of nucleotides, dictates everything from physical traits to cellular functions.
- Information Encoding:
The sequence of bases—adenine, cytosine, guanine, and thymine—forms a code. This code is read during transcription and translation, allowing cells to produce the proteins necessary for life. - Stability Through Structure:
The antiparallel strands and hydrogen bonding contribute to DNA's stability, ensuring that the genetic code is preserved through generations. Errors in these processes can lead to mutations, which can have significant consequences for organisms. - Storage Density:
The way DNA packs itself into chromosomes means that vast amounts of information exist within the confines of a tiny nucleus. This organization maximizes space while also allowing for efficient replication and repair mechanisms.
The ability to store genetic information accurately ensures that it can be passed down through generations, forming the basis of heredity. Any disruption or alteration of this structure can have cascading effects, impacting the organism in ways that can be seen or even at the biochemical levels.
Influence on Protein Synthesis
Another critical implication of DNA structure is its role in protein synthesis. Your body relies on proteins to function, from enzymes that catalyze biochemical reactions to structural proteins that provide support.
- Transcription:
The process begins with transcription, where the DNA sequence of a gene is copied into messenger RNA (mRNA). The delicate balance of the double helix unwinding and stabilizing ensures the accuracy of this step. Each base pairs with a complementary base on the mRNA, providing a faithful transposition of the genetic code. - Translation:
Following transcription, the mRNA strands are transported to ribosomes, where translation occurs. Here, ribosomes read the sequence and, by interpreting the codon language, synthesize proteins using transfer RNA (tRNA). The structure of DNA ensures that the message remains unaltered during this transition, preserving its intent. - Regulatory Mechanisms:
Transcription factors and other regulatory proteins interact with DNA at specific sites, which can turn genes on or off. The spatial arrangement and chemical modifications of DNA affect these interactions, driving how proteins are expressed in different cells and circumstances.
By maintaining an intricate dance of stability and flexibility within its structure, DNA not only encodes genetic information but also translates it into functional products through a highly regulated flow of information. Each twist of the helix is more than just aesthetic; it is a functional necessity that drives life as we know it.
"Understanding DNA structure is essential, as it provides the blueprint for the vast universe of biological diversity that surrounds us."
With that in mind, the implications of DNA structure are foundational not just for understanding how life operates, but also for advances in biotechnology, medicine, and genetic engineering.
The exploration of these functional implications deepens our grasp of both the underlying principles of biology and the potential manipulations that can be made to harness this knowledge for broader purposes.
Experimental Techniques for Analyzing DNA Structure
Understanding the intricate structure of DNA is crucial for insights into its function in biological systems. Numerous experimental techniques have emerged to dissect and visualize DNA, each bringing unique advantages and considerations. By amplifying our grasp of DNA's structure, these methods help illuminate its roles in genetics, heredity, and cellular processes. What follows is a deep dive into several key techniques that have significantly advanced our knowledge of DNA structure.
X-ray Crystallography
X-ray crystallography stands out as a technique that provides high-resolution images of molecular structures. The basic premise involves crystallizing a DNA molecule and then bombarding it with X-rays. This yields a diffraction pattern which, when interpreted, reveals the arrangement of atoms in the DNA.
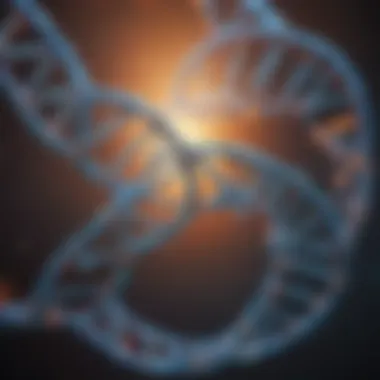
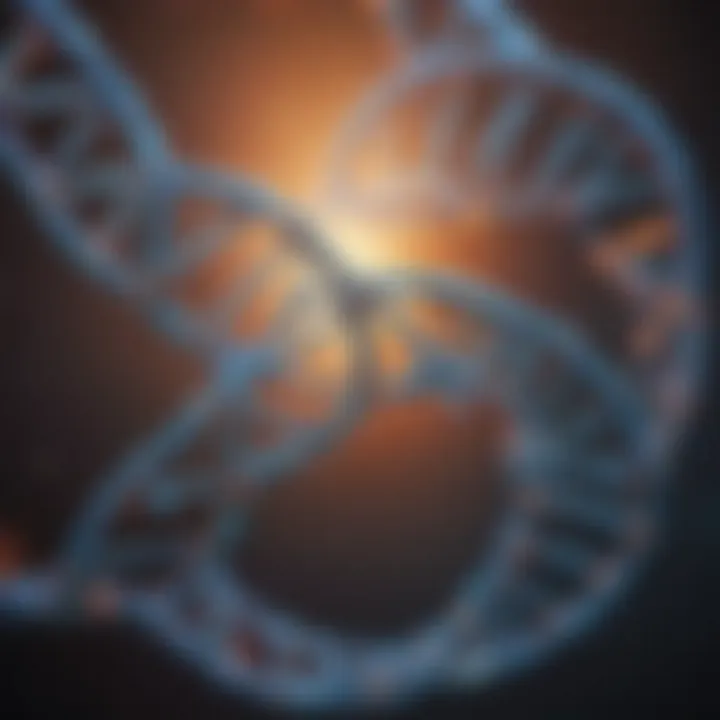
One remarkable advantage of this method is its ability to produce exceptionally detailed information about molecular geometry. For instance, when Rosalind Franklin employed this technique, her famous Photo 51 showcased the double helix structure of DNA, a turning point in molecular biology.
However, crystallization can be tricky; not all DNA segments form suitable crystals easily. It often requires specific conditions, thus may limit the study of certain DNA forms. Nonetheless, the detailed information X-ray crystallography provides cannot be overlooked, especially when it comes to understanding binding sites for proteins and drugs.
Nuclear Magnetic Resonance (NMR)
Nuclear magnetic resonance, or NMR, applies a different approach. This technique revolves around placing DNA in a strong magnetic field and measuring the absorption of electromagnetic radiation. By doing so, NMR can glean insights into the arrangement and dynamics of nucleotides.
The beauty of NMR lies in its ability to study DNA in solution, which is closer to its natural environment compared to techniques like X-ray crystallography. This helps researchers observe dynamic processes like base pairing and protein interactions. It has even been instrumental in determining the structures of complex DNA-protein assemblies.
Yet, like any method, NMR has its constraints; it is particularly suited for small to medium-sized DNA strands. A general rule of thumb is that as the size of the DNA increases, the spectra become increasingly complex and harder to analyze. Thus, while NMR provides valuable insight into molecular flexibility and conformational changes, it is not always feasible for larger constructs.
Cryo-Electron Microscopy
Cryo-electron microscopy (Cryo-EM) has made waves in recent years as a pioneering technique that captures biomolecules in their functional state. By rapidly freezing samples at extremely low temperatures, Cryo-EM preserves the structure and prevents ice crystallization. This method generates a 3D reconstruction based on electron scattering from the DNA molecules, providing high-resolution images that are invaluable.
The technique’s standout feature is its versatility; it's not limited to rigid structures but can also visualize dynamic and transient forms of DNA. This opens the door to studying various DNA configurations and their interactions under physiological conditions.
However, interpreting the resulting images can present challenges, as it often requires sophisticated computational techniques. Despite this, the clarity and realism achieved through Cryo-EM are unmatched, offering insights that improve our understanding of DNA's role within the broader context of cellular machinery.
The evolution of these experimental techniques grants researchers ample tools to dissect the complex nature of DNA, driving forward our comprehension of molecular biology and genetics.
DNA Replication
DNA replication is a fundamental process that ensures the genetic material gets accurately duplicated before a cell divides. This mechanism is vital for growth, repair, and reproduction in living organisms. Without DNA replication, the continuity of genetic information would be compromised, leading to cellular dysfunction and potential loss of organismal integrity. Therefore, understanding how DNA is replicated is a cornerstone of molecular biology and genetics.
Mechanism of Replication
The actual process of DNA replication is intricate, involving several stages and coordination between multiple components. At its core, the replication mechanism follows a semi-conservative model, meaning each new double helix consists of one original strand and one newly synthesized strand. This model is essential for maintaining genetic fidelity because it allows for the checking of errors during synthesis.
- Initiation: The process starts at specific locations in the DNA known as origins of replication. Here, the double helix unwinds, creating a replication bubble. Enzymes called helicases are critical during this phase as they separate the two strands of DNA, allowing access to the bases needed for copying.
- Elongation: Once the strands are separated, another enzyme, DNA polymerase, begins synthesizing a new strand. It adds nucleotides complementary to the template strand—adenine pairs with thymine, and guanine pairs with cytosine.The synthesis occurs in a 5’ to 3’ direction. One of the striking elements here is that while one strand (the leading strand) is synthesized continuously, the other strand (the lagging strand) is made in small segments called Okazaki fragments. This need for careful management highlights the complexity of the replication machinery.
- Termination: Finally, when the entire DNA molecule has been replicated, the replication process concludes. Enzymes like ligase play a crucial role in sealing gaps between fragments on the lagging strand, ensuring a complete and continuous DNA molecule.
Role of Enzymes in Replication
Enzymes are the workhorses of DNA replication, each serving a specific function to facilitate the accurate and efficient copying of genetic material. The main players in this complex ballet are DNA polymerase, helicase, and ligase. Each contributes dramatically to the replication process, and their characteristics determine how effectively DNA replication occurs.
DNA Polymerase
DNA polymerase is perhaps the star of the replication show. Its main function is to add nucleotides to the growing DNA strand, ensuring the accurate matching of bases according to the template. One characteristic that stands out is its proofreading ability, as this enzyme can detect mismatched bases and correct them in real-time. This function is crucial to maintaining high fidelity during replication.
What makes DNA polymerase especially beneficial in scientific research and clinical applications is its high specificity and speed. These traits make it a popular choice in PCR (Polymerase Chain Reaction) and other molecular techniques. However, its activity is restricted to a specific set of conditions, as it's sensitive to changes in temperature and pH, which may limit its effectiveness in certain environments.
Helicase
Helicase is another critical enzyme in the replication process. Its primary role is to unwind the double-stranded DNA, allowing the two strands to separate. This enzyme takes the lead in creating the replication fork, a structure essential for DNA replication to occur. A key characteristic of helicase is its energy dependence; it requires ATP (adenosine triphosphate) to function properly.
The unique feature of helicase lies in its ability to rapidly unzip the DNA helix, enabling simultaneous replication of both strands. However, this high-speed function can also be a double-edged sword, as it may potentially lead to strand breaks if not properly regulated.
Ligase
Ligase serves a vital yet often overlooked role at the final stages of DNA replication. Its primary job is to connect the Okazaki fragments on the lagging strand, which ensures a continuous helix. A standout characteristic of ligase is its ability to form covalent bonds between the sugar-phosphate backbone of adjacent fragments, effectively sealing the gaps to form a coherent strand.
The unique contribution of ligase is its function in maintaining DNA integrity, especially in the context of damage repair processes during replication. Nonetheless, its activity is constrained by the need for specific ions, which can sometimes create limitations in its effectiveness under certain molecular conditions.
"Preservation of the genetic code requires precision; enzymes enacting DNA replication reveal a narrative of coordination and specialization in biology."
In summary, the mechanics of DNA replication underscore the intricate relationship between structure and function in genetics. Understanding these processes not only enhances our grasp of molecular mechanics but also inspires potential biotechnological applications, reinforcing the significance of DNA in life as we know it.
DNA Repair Mechanisms
DNA repair mechanisms hold a significant position in the larger context of molecular biology. They are essential for maintaining the integrity of genetic information throughout an organism’s lifetime. The DNA structure is susceptible to various types of damage caused by environmental factors, metabolic processes, and replication errors. When these damages occur, it is crucial to repair them efficiently to prevent mutations that might lead to diseases, including cancer. Understanding DNA repair mechanisms provides insights into how organisms evolve, adapt, and maintain their genetic information over generations.
Types of DNA Damage
DNA can be harmed in numerous ways, and understanding these mechanisms of damage is key to comprehending how cellular repair systems function. Damage can generally be classified into several categories:
- Single-strand breaks (SSBs): These occur when the phosphodiester backbone of the DNA is broken at one site. Environmental factors like radiation or oxidative stress can cause these breaks.
- Double-strand breaks (DSBs): More severe than single-strand breaks, these involve damage to both strands of the DNA helix. These issues can arise from ionizing radiation or certain chemicals.
- Base modifications: Chemicals or environmental stresses might alter the structure of the nitrogenous bases in DNA. This can lead to mispairing during replication.
- Cross-linking: This occurs when DNA strands are linked together by covalent bonds due to certain chemicals or environmental influences, hindering replication and transcription.
Repair Pathways
The cell has evolved multiple pathways to mend various types of DNA damage effectively. Each pathway is specialized to address specific lesions, ensuring the accuracy and stability of the genome. Here are three key pathways:
Nucleotide Excision Repair
Nucleotide Excision Repair (NER) is invaluable in handling bulky DNA lesions that distort the double helix structure, such as those introduced by UV radiation. This pathway identifies and excises a section of damaged DNA, allowing for the synthesis of a new strand using the undamaged complementary strand as a template. A notable feature of NER is its ability to repair a diverse array of damage types. It provides a broad-spectrum solution that is particularly beneficial for the organism’s survival. However, this pathway can be slow since it involves several steps, including damage recognition, strand excision, and DNA synthesis. If the damage is too copious, this process might not keep up with the rate of damage accumulation, which poses a risk of genome instability.
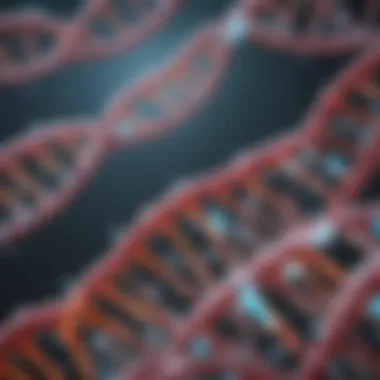
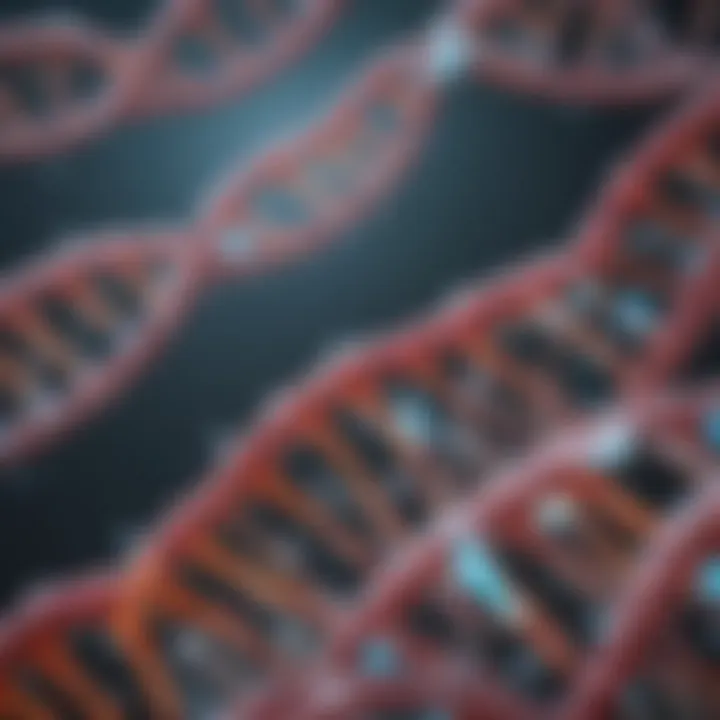
Base Excision Repair
Base Excision Repair (BER) targets non-helix-distorting base lesions caused by oxidation, deamination, or alkylation. In this pathway, specific DNA glycosylases recognize and remove the damaged base, creating an abasic site. The sugar-phosphate backbone is then cleaved by an endonuclease, allowing DNA polymerase to fill in the gap and DNA ligase to seal the nick. A key characteristic of BER is its precision in repairing single base alterations, which makes it highly efficient for minor repairs. While it efficiently manages small variations, it is less effective against more significant forms of damage, such as double-strand breaks.
Mismatch Repair
Mismatch Repair (MMR) is critical for correcting errors that arise during DNA replication, especially those involving base-pair mismatches and insertion-deletion loops. This pathway recognizes and repairs incorrectly paired nucleotides by directing proteins to the incorrect base, which is then removed and replaced with the correct nucleotide. A central feature of MMR is its role in the prevention of mutations during DNA replication, thereby maintaining the genetic stability of the organism. However, because it operates during DNA synthesis, its effectiveness can sometimes be hampered by the speed of replication; if too many mismatches occur quickly, this pathway may struggle to keep pace.
DNA repair mechanisms are the unsung heroes in the story of genetic integrity, ensuring that the blueprint of life remains intact.
These repair pathways indicate the complexity and adaptability of DNA repair systems within cells. They not only sustain genetic integrity but also present potential targets for therapeutic interventions, aimed at treating genetic disorders and cancers, making them vital topics of research in molecular biology.
Functional Variations in DNA Structure
Understanding the functional variations in DNA structure is essential as these nuances can significantly influence genetic activity and expression. While the classic double helix is the model most commonly associated with DNA, the existence of alternative structural forms serves critical biological functions. These variations can play a role in genetic stability, replication, and gene expression, providing a deeper insight into the intricacies of molecular biology.
Supercoiling
Supercoiling is a fascinating twist on the classical structure of DNA. It refers to the extra turns of the helix that go beyond the normal twist of the double helix. Think of it as modifying a coil — when you tighten a coil, you store energy, and something similar happens in DNA. Supercoiling can either be positive or negative, with positive supercoiling introducing additional turns, while negative supercoiling unwinds the DNA, which can be vital during transcription and replication.
This structural variation is crucial because it aids in compaction of DNA within the cell's nucleus, ensuring that it fits neatly within the tight confines while maintaining accessibility for necessary cellular processes. Essentially, it’s like coiling a rope to keep things tidy.
Alternative DNA Structures
There are several notable alternative structures to consider:
Z-DNA
Z-DNA is a left-handed DNA helix, which contrasts with the right-handed twist of the canonical double helix. The key characteristic of Z-DNA is its zigzag backbone, which emerges under certain conditions, such as high salt concentrations or negative supercoiling. This unique feature contributes to its biological significance, particularly in regions of active transcription. Some studies even suggest its involvement in gene regulation and potential interactions with proteins that influence gene expression. Its less stable nature, however, raises questions about its role in sustained genetic contexts, making it a curious subject for ongoing research.
A-DNA
A-DNA is another alternative that takes a different approach altogether, especially in dehydrated conditions. This right-handed helix is more compact than B-DNA, and its key characteristic is a tighter helical screw sense. This compact form can be advantageous in scenarios like specific molecular interactions or during protein synthesis. However, it doesn’t tend to play a significant role in standard genomic integrity, which is why it’s less frequently discussed compared to its cousins.
H-DNA
H-DNA, or triplex DNA, comprises three strands instead of the usual two. This unique version tends to form in regions with repeated sequences, such as telomeres or regulatory regions. Its defining attribute is its capacity to hold onto more information due to an extra strand. H-DNA might provide some advantages in terms of regulatory capacity; however, its stability poses challenges that merit more careful consideration in potential applications.
Alternative DNA structures remind us that the genetic landscape is not as straightforward as initially presumed. Each form harbors potentialities that could lead to breakthroughs in understanding genetic functions.
In summation, functional variations in DNA structure play instrumental roles in genetic functioning. Understanding these variations — supercoiling and alternative formations like Z-DNA, A-DNA, and H-DNA — leads to richer insights on genetic regulation and stability, calling attention to the importance of structural diversity in the DNA helix.
Implications of Structural Diversity
The concept of structural diversity within DNA is not just a theoretical notion—it bears significant weight in genetic functioning and cellular processes. Each twist and turn of the DNA molecule can have profound implications on how genes are expressed, regulated, and ultimately, how they contribute to the complexity of life. Given this complexity, it’s crucial to underscore some of the fundamental aspects regarding structural diversity.
Genomic Stability
Genomic stability is paramount for the survival of organisms, as it ensures that the genetic code remains intact over generations. Structural variations within DNA—like supercoiling or the formation of alternative DNA structures such as Z-DNA—play pivotal roles in maintaining this stability. For instance, supercoiled DNA in bacteria helps in packaging the genome into a compact form, thus protecting it from damage and ensuring smooth replication.
Furthermore, improper structural configurations can lead to genomic instability, which is often a precursor to diseases, including various forms of cancer. Understanding these structural nuances can aid in developing therapies aimed at stabilizing the genome, ultimately enhancing cellular health and longevity.
Gene Expression Regulation
Another arena where structural diversity exhibits its influence is gene expression regulation. The accessibility of DNA is tightly linked to its structural configuration. For instance, when DNA is tightly coiled, gene expression is generally suppressed. However, when chromatin structures relax, the associated genes become accessible for transcription, which means they can be read and translated into proteins.
A variety of proteins, termed "transcription factors," interact with specific structures of DNA to either promote or inhibit transcription. This means that the subtle changes in DNA’s architecture can lead to drastically different outcomes in gene expression. Moreover, factors such as methylation and acetylation alter the conformation of DNA, influencing the binding affinity of transcription machinery.
The flexibility and adaptability in DNA structure are integral for the fine-tuning of gene expression, allowing organisms to respond to environmental changes effectively.
In summation, structural diversity within DNA is not merely an academic interest; it bears tangible consequences on genomic stability and the regulation of gene expression. The interrelationship of these concepts underscores the importance of studying DNA's structural implications within the broader context of molecular biology and genetics. The exploration into how these structures dictate functionality continues to be a fertile ground for research, offering insights that can revolutionize approaches to treating genetic disorders and understanding life at a molecular level.
Finales
In exploring the multifaceted world of DNA's chemical structure, this article delineates the intricate dance of molecules that hold the very blueprint of life. Understanding DNA is not just a scientific pursuit; it's about grasping the essence of heredity, variation, and biological function itself. As we draw near the conclusion of this analysis, it becomes evident that the implications of DNA's structure extend far beyond textbooks and walls of laboratories.
Summary of Key Insights
The investigation into DNA has brought to light several pivotal insights:
- Chemical Composition: The basic unit of DNA, the nucleotide, consists of a sugar, phosphate, and a nitrogenous base. It is the unique sequence of these nucleotides that defines genetic information.
- Structural Harmony: The double helix structure is not merely a physical formation; it promotes stability and efficient replication mechanisms crucial for cellular function.
- Hydrogen Bonds: This seemingly fragile interaction between complementary bases is responsible for the fidelity of genetic replication and transmission.
- Functional Relevance: The chemical architecture of DNA significantly influences gene expression, impacting everything from cellular responses to evolutionary adaptations.
- Experimental Techniques: Methods like X-ray crystallography and NMR have been instrumental in deciphering the dynamic structure of DNA, revealing insights that were once the stuff of speculation.
These insights underscore why DNA research remains a cornerstone of modern biology and genetics. Understanding these concepts enables scientists and educators alike to unravel mysteries of various biological phenomena, potentially leading to breakthroughs in medicine and evolutionary biology.
Future Directions in DNA Research
As we look ahead, the field of DNA research is poised for remarkable advancement. Here are some noteworthy avenues that merit attention:
- CRISPR Technology: As gene-editing tools evolve, the implications of altering DNA sequences raise ethical considerations alongside scientific inquiries.
- Synthetic Biology: The construction of artificial DNA molecules opens doors to novel biological functions and could revolutionize the production of pharmaceuticals and biofuels.
- Epigenetics: Understanding how environmental factors influence gene expression through epigenetic modifications can reshape our approach to diseases and health.
- Computational Biology: With the rise of big data, machine learning is expected to play a pivotal role in predicting DNA structure and function based on massive genomic datasets.
- Personalized Medicine: The ability to tailor medical treatments to individuals based on their unique DNA sequences holds potential for more effective healthcare solutions.
"The journey into the complexities of DNA is one of continual discovery, a testament to nature's elegance and complexity."
In summary, the research surrounding DNA’s structure and function is a field in constant evolution, one that feeds the insatiable quest for knowledge about life itself. As new technologies emerge and our understanding deepens, the study of DNA will only become more pivotal in addressing the challenges faced in biology and medicine today.